Nearly five years on from the first Gravitational Wave detections which verified a 100-year-old prediction of Albert Einstein’s General Theory of Relativity, advances in Gravitational Wave astronomy are propelling us to newer horizons.
The first direct observation of a Gravitational Wave event, GW150914 (short for-Gravitational Wave event observed on 2015-09-14), announced by the LIGO and Virgo collaborations on 11 February 2016, was one of the most significant moments of the previous decade in Physics. Decades of worldwide effort towards building and operating the extremely sensitive detectors had confirmed the existence of Gravitational Waves.
Dr. Astrid Lamberts, CNRS researcher at Observatoire de la Côte d'Azur, Nice, France, recalls the events of 2015.
“When the first detections happened, it completely blew my mind. The idea of 30 solar mass black holes was completely unexpected. I had worked in labs with many members from LIGO, so I had heard of it. But thinking about this after the first detection just made me completely switch fields, and I started working on population models for the binary systems.”
After a successful first observational run (O1), LIGO was shut down for upgrades and started operations again (O2) in 2017 as Advanced LIGO, located at Livingston and Hanford, USA, along with Advanced Virgo in Cascina, Italy. The third observational run (O3) began in April 2019 and the three detectors were online till March 2020, when operations had to be shut down due to the COVID-19 pandemic.
It was during O3 that the unprecedented detection of Neutron Star-Black hole coalescence has been made.
The latest results
Two systems: GW200105, with component masses 8.9 and 1.9 MO, and GW200115, with component masses 5.7 and 1.5 MO (where MO is equivalent to the mass of the Sun) were observed in O3 with a 90% credibility level. These are the first detections in which the mass of the primary object was greater than the minimum mass ever observed for Black-Holes, i.e. 5 MO, and the secondary’s mass was less than the maximum observed mass for a neutron star, i.e.. 2.3 MO, leading to the conclusion that these Gravitational Wave signals came from mergers of Neutron Star-Black Hole binaries.
Dr. Lamberts led the team that authored the paper by the LIGO, Virgo, and KAGRA collaborations announcing the detection of these Neutron Star-Black Hole systems.
“It's a new type of system that we have detected. We had never observed Neutron Star-Black Hole systems before. So, knowing that they actually exist is a big thing. There had been many theoretical predictions that Neutron Star-Black Hole mergers exist, and it's not a surprise. But being able to make a measurement and then infer the merger rate, this is the very first time.” she says.
Binary Evolution and Coalescence
Compact object binaries, which include Binary Neutron Stars (a system having two Neutron stars revolving around each other), Binary Black holes, and Neutron Star-Black Hole binaries, can form through several stellar evolution channels.
Isolated binaries are formed when two massive stars are born and evolve next to each other to eventually result in a binary system of compact objects, like Neutron Stars or Black Holes. As the two objects revolve around each other, they emit Gravitational Waves.
Einstein’s General Theory of Relativity interprets Gravity as distortions in the fabric of Space-time. These distortions travel away from the region of generation in a fashion like ripples moving outwards on the surface of the water. The waves carry energy away from the binary system, reducing its angular momentum. Eventually, after millions of years of losing energy, the compact objects collide, producing a sumptuous final wave, which can be detected at Earth-based Gravitational Wave detectors.
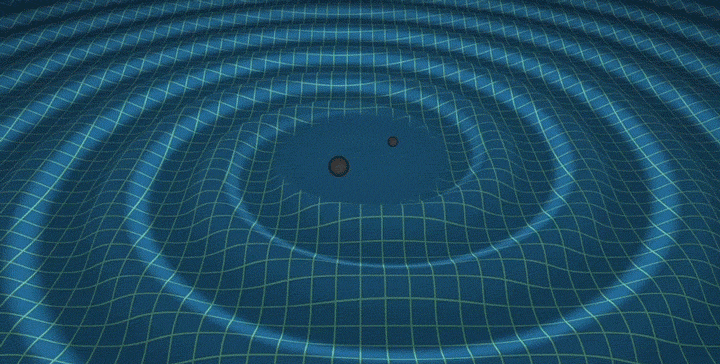
“We currently have a detector that measures kilohertz systems. These are systems that rotate around each other about 1000 times per second. So obviously, we're talking only about really compact things because they have to be able to get so close to each other that they are about to merge. This is why we see neutron star-black hole mergers.” says Dr. Lamberts.
The Detector
The technology for detecting gravitational waves had been in development for over half a century before the first detections in 2015.
Prof. Livia Conti, an experimental physicist at Istituto Nazionale di Fisica Nucleare (INFN), Italy, describes early efforts towards detecting the elusive Waves.
“Joe Weber, in the early 1960s at the University of Maryland, started working on this idea of detecting gravitational waves, which was very crazy at the time and the instrument that he designed was an acoustic detector. He wanted to measure the vibrations of a metal piece when the Gravitational Wave passes by and gives some energy to the metal. It was older technology, and at some point, was dismissed, and now interferometers are used to detect Gravitational Waves. They are much better suited to detecting them.”
“The interferometer, with this ‘L’ shape is perfectly designed for this purpose. We measure the difference in the time that light takes to travel the two perpendicular arms, by observing the interference pattern when the two light beams recombine after traveling forth and back along the arms, which are equal in length(3 and 4 kilometers for Virgo and LIGO respectively). When there is no Gravitational Wave signal you have perfect destructive interference. So, you measure no light, we say the interferometer output is a dark fringe. As a Gravitational Wave passes through the interferometer, it produces a differential change in the length of the two arms (at some instance by stretching one arm and shrinking the other, and then vice-versa), which causes some constructive interference. So, the interference pattern at the detector port changes: the interferometer output is not dark anymore. The photodiodes then produce a signal proportional to the incident light” she added.
LIGO, Virgo, and KAGRA detectors rely on these minuscule changes in the arm length (of the order of 10^-18 m) to detect warping of Space-time as a Gravitational Wave passes through the detector.
“To be able to detect waves with a frequency of about 100Hz, you would need arms that are about 700 kilometers in length,” says Prof. Conti. “It is, of course, not feasible to construct such detectors on Earth. To overcome this, we place an additional mirror next to the beam splitter, at the beginning of each arm. These mirrors reflect the light in the arms, back and forth, and increase the time the light spends in each arm. We effectively increase the distance traveled by light before recombining at the beam splitter and maximize the signal.”
Along with increasing the sensitivity of the detector, this technique also helps dilute the biggest source of inconvenience in Gravitational astronomy: Noise.
Noise
Along with Gravitational Wave signals, interferometers pick up substantial amounts of unwanted disturbances, called noise. This can be due to fundamental error sources like the Uncertainty Principle, the thermal agitation of the atoms constituting the mirrors and their suspension, technological reasons like stray light, and even the simple fact that the detectors are situated on Earth- Seismic noise. Each source affects different frequency bands of the detector.
“Apart from the main channel that samples the photodiode at the detector output and eventually measures the passage of a Gravitational Wave, you have about 200,000 auxiliary channels that measure different quantities related to the external and internal environment of the detector. We also measure disturbances due to human activities like control systems, electric mains, and even the air conditioners,” says Prof. Conti. “‘Coherence’ between the main channel and any of the auxiliary channels, is indicative of their coupling. Such occurrences need to be avoided. However, if some residual coupling exists, it should be measured so that we can subtract the noise or at least take it into account.”
“Noise at higher frequencies is dominated by the shot noise, the noise incurred while counting the photons. We reduce its significance by increasing the power of the laser source. The signal-to-noise ratio is inversely related to (the square root of) the power... We even use a quantum technique called squeezing to shape the uncertainty in the photon counting noise and its counterpart, the noise in the radiation pressure on the mirrors, to further reduce the effect of quantum noises: squeezing has been in use at both LIGOs and Virgo during O3. ” says Prof. Conti.
“In the middle frequency, you have thermal noise. This is the Brownian motion of the atoms of the mirror (that is, the transparent substrate and the reflective coating). These vibrations can mask the tiny displacement caused by the passing of gravitational waves and limit the sensitivity in the middle-frequency range.” she said.
Another source of noise that is quite irksome and causes several glitches is what is described as stray light.
“A small fraction of the laser light exits the main path and is lost. If not absorbed, it may hit a (partially) reflecting surface and re-enter the main path, bringing in the phase noise due to the vibrations of the reflecting surface. This is also a big problem," said Prof. Conti
“At low frequency, we have seismic noise, which is due to the vibration of the ground because, at the end of the day, everything is supported on Earth. You are mainly concerned by the noise caused by the horizontal motion of the Earth’s crust. But not only that: the degrees of freedom are coupled because the Earth’s local gravity is not parallel at the two mirrors in the same arm, as they are some kilometers apart. Therefore, we must reduce the seismic noise in all degrees of freedom. Virgo has mirrors which are suspended by 10-meter-long chains of pendula and cantilevers which dampen the effects of the Earth's vibrations and limit the seismic noise.” she added.
Once the data is recorded they need to be calibrated; then some noises can be subtracted and some noisy time periods are vetoed. The data is then processed to search for astrophysical signals and extract physical information about the Event.
Analysis
The data collected by the detectors is riddled with noise, with only a small fraction being Gravitational Wave signals. The first task, then, is to check if the data contains a signal, and if it does, isolating the signal and drawing physical inferences from the same.
“There are essentially two ways of looking for the Gravitational Wave signal,” states Dr. Stefano Bagnasco, Senior Technology Researcher at Istituto Nazionale di Fisica Nucleare (INFN), Italy. “The first exploits the fact that you have a network of detectors and you can find coherent signals in a set of them.”
Thus, if we find a strong ‘correlation’ between the data collected from multiple observatories, we can use the same to isolate the signal. In this context, correlation is quantified as a mathematical parameter defined for 2 signals over the same independent variable i.e., Space-time. This way we can look for unexpected phenomena that cannot be modeled theoretically.
“The second method gives better results for compact binary coalescences” adds Dr. Bagnasco. “You need to have some knowledge of physics that governs the process so that you can simulate it and compute signal templates (expected waveforms), banks of signal templates, calculated using Numerical Relativity. Then what you do is you try to superimpose these on the data coming from the experiment until you find a match,” explains Dr. Bagnasco.
This method is called Matched Filtering.
Each of the aforementioned templates has its specific parameters which are assumed to be random variables. These parameters represent the binaries’ masses and spins. The total mass of the binary system, also known as chirp mass, is first established by comparing the templates with the detected signals. Individual probabilities of the parameters belonging to that specific Gravitational Wave signal are then determined using Bayes’ Rule of Probability. The parameters with maximum probability are selected and are eventually associated with that particular Gravitational wave event.
“The in-spiral signal, the one that we measure is this wave that increases in amplitude and also in frequency. This is decided by the chirp mass, the combination of the two masses. Thus, we measure chirp mass very well, while the individual mass measurements have larger errors.” explains Prof. Conti.
The Neutron Star-Black Hole mergers announced on 1 July 2021, set the probability that the secondary’s mass is below the maximal mass of a neutron star is 89%–96% and 87%–98%, respectively, for GW200105 and GW200115.
These detections have thus proven to be a giant step ahead in Gravitational Wave astronomy and researchers look forward to detecting many more exotic mergers in the future.
The Future of Gravitational Wave Astronomy
Post O3, LIGO, Virgo, and KAGRA have been shut down for upgrades.
“Improving the instruments basically means reducing the noise. We are increasing the laser power to lower the shot noise. We are complicating the optical scheme of the interferometer by adding frequency-dependent squeezing in all detectors. In Virgo, this is being done by adding a signal recycling mirror, which is a reflecting mirror between the beam splitter and the photodiodes. This mirror will reflect the sidebands induced by the passing gravitational waves before they are detected to maximize the strength of the signal. Signal recycling is already present in LIGO. Phase noise due to stray light is an emerging issue in both LIGO and Virgo, and there is a lot of work happening to reduce this.” said Prof. Conti.
Gravitational Wave astronomy is at its beginnings. Following the success of LIGO and Virgo, many future detectors are under work. The most highly anticipated of these is European Space Agency’s space probe LISA (Laser Interferometer Space Antenna).
“LISA is going to be a millihertz detector. This means we can look at the merger of larger objects. LISA will see mergers of supermassive black holes, the ones that live in the center of galaxies. They are of 105-106 solar masses and they merge at low frequencies, right in the LISA band,” explains Dr. Lamberts.
“We will be able to see white dwarf binaries in the Milky Way or very nearby galaxies as they simply turn around each other.”
“LISA will also see a few sources that LIGO will see a few years later. So, if you have a big enough Black hole like the first gravitational wave event, a 30 solar mass black hole, LISA would have been able to see it a few years before LIGO when the black holes were just turning around each other and the system was close enough and loud enough.”
LISA is currently scheduled to go online in the 2030s and will provide a completely new window to discover more about the Universe.
Dr. Lamberts is optimistic about the future of the field.
“I'm just excited to see what's coming next. It's a whole new field of astronomy. The way we think about massive stars has, in my opinion, has really changed since we have seen remnants that are more massive than we expected. Mass ratios that are strange, spins that are puzzling us, anomalous detections that don’t fit in with the theory... We have more questions than answers and it's definitely exciting.”
Note: Readers interested in the computational architecture behind Gravitational Waves detection should look out for our November Issue, where the experimental effort towards detecting Gravitational Waves is explored through a computer scientists’ lens.